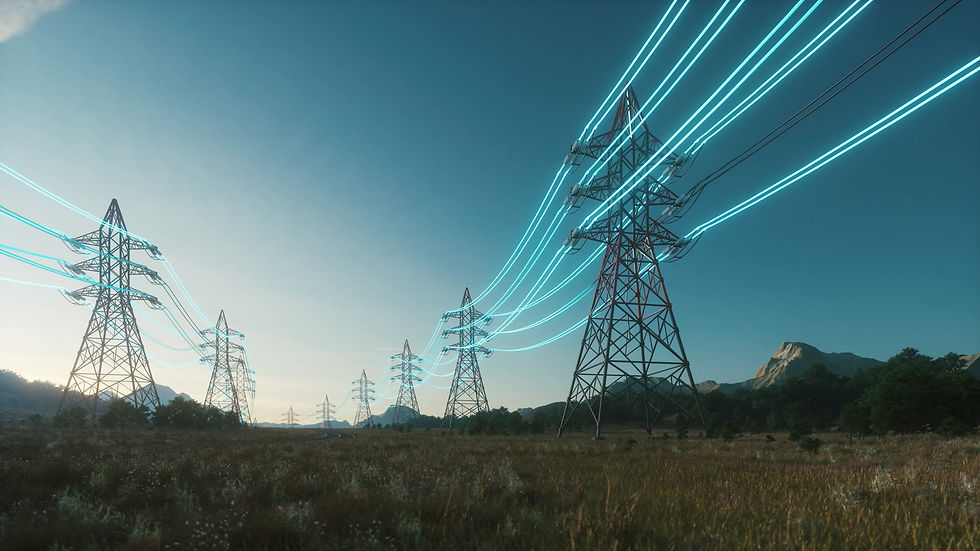
Executive Summary
This report investigates long-term strategies for achieving net-zero emissions across Europe by developing deep decarbonization pathways. These pathways are detailed technical plans on how Europe can transform the economy to meet emissions reduction targets, created using a model that optimizes investments in and operations of the energy system. We generate multiple pathways under varying situation assumptions to provide an extensive look at how Europe can reach net zero. This report interprets these highly technical and granular analyses into a cohesive narrative with viable actions for European policymakers, industry, and civil society. Our approach complements the European Commission's traditional policy projection models, which emphasize the potential impact of current policy, by offering a dynamic perspective on the risks and opportunities of a wide range of emission’s reduction strategies.
Prescriptive Policy
In recent years, the EU has made significant advancements in its climate policy, driven by the comprehensive Green Deal agenda. The EU has established a legally binding target to achieve climate neutrality by 2050, aiming to reduce greenhouse gas emissions by at least 55% by 2030, relative to 1990 levels. To achieve this ambitious goal, the “Fit for 55” package was introduced, comprising a series of legislative proposals intended to transform key sectors such as energy, transportation, and industry. This package includes critical reforms such as bolstering the EU Emissions Trading System (ETS), introducing carbon pricing for traditionally exempt sectors like shipping and aviation, and rapidly scaling up renewable energy deployment across all EU Member States. Collectively, these measures form the backbone of the EU’s strategy to cut emissions and promote a low-carbon economy.​​​​​​​​​​​​​​​​
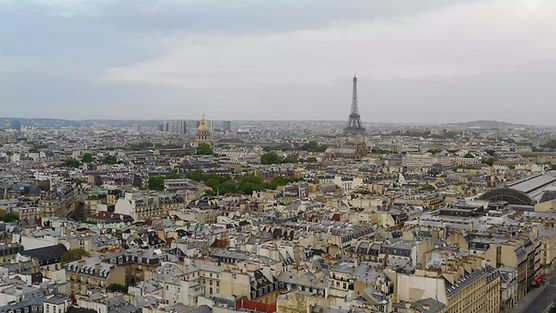
​​​​However, while sector- and technology-specific targets are essential to guiding member states toward meeting these climate objectives, there are challenges with the current approach. These targets, though not always legally binding, serve as important indicators of progress, yet they often lack the flexibility necessary for effective decarbonization at the national level. Member states may struggle to implement them through their own climate policies if the targets are overly aggressive or rigid. Additionally, technology-specific targets may overlook complex market realities and supply chain issues, creating the false perception that there is a single, uniform pathway to net zero. It is crucial that the EU balances ambition with adaptability, ensuring that its targets are robust yet flexible enough to accommodate diverse national circumstances and the evolving challenges of the green transition.
​
While recognizing the need for market transformation, we specifically question the feasibility of some of the ambitions for clean hydrogen and sequestration in the near term. Supply targets for clean hydrogen outstrip demand in 2030 as use cases in transport and industry are still developing and the principal user of hydrogen today–petroleum refineries–is in decline. Similarly, 2030 sequestration targets have higher than expected deployment of carbon capture technologies. While these 2030 targets are likely more than what is required within the next six years to stay on track for carbon neutrality, these technologies are essential for reaching net zero, and policymakers are right to support creating early markets for their deployment but should also be realistic about near-term prospects.
​
Longer-term targets are generally the right level of ambition, though they are not de facto appropriate in scale, and achievement of some of the targets is highly dependent on choices made elsewhere in the energy system. As policymakers look toward establishing 2040 targets, it is critical to understand where these targets do and do not reflect success in emissions reduction goals and provide EU Member States with a more flexible framework that accounts for the diversity of potential strategies beyond 2030.
The Cost of Inaction
The transformation of the energy system undeniably raises financial concerns for both consumers and governments; however, if executed properly, the journey toward a decarbonized Europe—and the outcome—will ultimately lead to cost savings. In this year’s ADP, we examined not only net-zero Scenarios but also a Baseline Scenario in which consumer technologies were frozen at their 2021 levels, and no carbon policies were implemented to influence energy supply. This approach allows us to evaluate the full costs of decarbonization, including efficiency and electrification measures, which ultimately reduce costs for consumers.
​
Achieving a cost-effective transition, however, will require a rapid increase in the capital available. This includes energy investments; ongoing support for research, development, and deployment of key decarbonization technologies; and assistance for consumers with the upfront costs of more expensive vehicles, heating systems, and building upgrades that only deliver savings over time. It will necessitate establishing appropriate market signals to encourage rational private investment. Each of these goals is challenging on its own, and together they are even more demanding. Nevertheless, our analysis shows that the potential rewards are enormous: a decarbonized economy that saves money.
Winter Is Coming
In the long run, Europe is likely to face a distinctly winter-peaking electricity demand due to the widespread electrification of heating systems. This seasonal peak presents a significant challenge, as the periods of highest demand often coincide with some of the lowest renewable energy generation capacity, particularly during winter months. Solar energy, which is a key resource in many high-load countries, tends to contribute very little during these times, sometimes for sustained periods. This dynamic makes it difficult to rely solely on renewables during high-demand winter periods, and it highlights the complexity of meeting sustained high net-load events (demand minus renewable generation). It also underscores the need to move beyond simplistic resource comparisons based on metrics like Levelized Cost of Energy (LCOE) and to recognize that resource competitiveness will evolve as the grid and market conditions change.

To address these challenges, our results suggest the need for a diverse portfolio of energy resources. In addition to deploying hundreds of gigawatts of short-duration energy storage to manage daily fluctuations, there is a growing role for multi-day energy storage (MDES) systems, capable of providing power over several days when renewable output is low. An expanded nuclear fleet will provide baseload carbon-free power. Furthermore, up to 250 gigawatts of new backup generators—designed to be fuel-flexible (capable of running on fossil gas, hydrogen, or biogas) and running at less than 15% of the time—are necessary to ensure electricity reliability.
​
The figure below shows each resource's energy production during a winter reliability event (defined here as the day with the highest net-load across Europe) as well as an example summer day. This illuminates that despite huge increases in renewable capacity, the reliability events are triggered during periods of their lowest production and thus will require complementary reliability resources. This is not a reason to forego renewables (our model chooses multiple terawatts of these resource economically) but it is an argument to be clear about what other types of resource will be necessary to support them.
Where the Wind Blows
This analysis underscores the critical importance of expanding Europe’s wind resources, with all Scenarios projecting over 900 GWs of combined onshore and offshore wind capacity by 2050. Although this capacity is expected to be lower than overall installed solar capacity, wind provides significantly more energy due to its higher capacity factors. Additionally, the energy generation profiles of wind offer more seasonal consistency than solar, making it a more reliable contributor to the energy mix during winter months when solar output is minimal. Even when assumptions regarding the resource potential of wind are drastically changed, the model compensates by increasing reliance on offshore wind, showcasing wind’s desirability in various geographical and resource conditions.
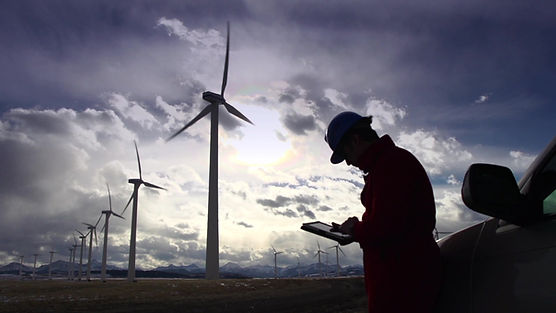
The pivot from onshore towards offshore wind would significantly alter the spatial distribution of wind resources across Europe. Unlike the aggregate totals, which may appear uniform, there is considerable variability in wind deployment at the national level. The determining factor in the expansion of wind resources lies in where they can be developed most cost-effectively. In the long-term, this geographic flexibility becomes crucial for supporting locationally agnostic electricity loads, such as electrolysis, industrial electrification, direct air capture, and possibly data centers and server loads. Wind resources developed in these optimal locations will help drive the future decarbonization of industries and facilitate Europe's transition to a cleaner energy system.
What to Do with the CO2
Achieving net-zero emissions necessitates a significant expansion of carbon capture technologies, with industry, biofuels, and direct air capture emerging as the primary opportunities in our modeling. However, the fate of the captured carbon remains uncertain and is contingent on a variety of factors including technological advancement, policy frameworks, and public acceptance. Captured carbon can either be sequestered in geological formations, such as depleted oil and gas fields, coal seams, or saline aquifers, or it can be utilized to produce substitutes for liquid and gaseous fossil fuels. The feasibility of each pathway depends on advancements in related technologies such as electrolysis, e-fuel synthesis, and the ability to securely inject and monitor carbon for sequestration.
​
In countries where sequestration is prioritized, geological conditions determine viability, with certain areas having more suitable formations for long-term carbon storage. Proximity to carbon capture sources is also a critical factor in determining the level of sequestration since shorter distances lower transportation costs. On the utilization side, the development of renewable resources plays a decisive role. The availability of surplus renewable energy—beyond what is needed for direct consumption—makes it possible to produce carbon-based fuels, thus making utilization more attractive in countries with abundant renewable energy potential. We don’t model the totality of carbon dioxide removal approaches that might be available due to a lack of data inputs on costs and potential, but pursuing these approaches might be able to reduce the amount of infrastructure devoted to things like DAC or provide additional emissions offsets benefits to the rest of the economy.
Electricity Market Evolution
Electricity markets are systems where electricity is bought and sold between generators and consumers, often mediated by market operators. These markets ensure the efficient transaction of electricity and set prices based on supply and demand dynamics. Traditionally, power generation has relied on hydro and nuclear energy as well as fossil fuels like coal and natural gas, but the growth of renewable energy sources like wind and solar has significantly impacted these markets. Unlike conventional power plants, renewables have no variable costs once installed, leading to them bidding into the market at very low or even negatives prices.
This influx of low-cost energy from renewables can drive down the overall market price of electricity. However, while the addition of more renewables can lower market prices, it also introduces new challenges concerning the reliability and stability of the power grid. To ensure a stable electricity supply, capacity resources like batteries, gas plants, and other dispatchable power sources are still needed to balance the grid during periods of low renewable output. The current market structures often undercompensate these capacity resources, as they are primarily paid for according to the energy they provide rather than their availability to meet peak demand. This highlights the need for market reforms that appropriately value and compensate the capacity resources for the crucial role they play in ensuring grid reliability, even as we transition to cleaner energy sources.​
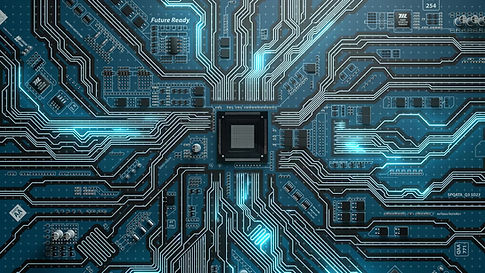
​If deployment of low-marginal cost generators outpaces electricity market reform, we could see the retirement of resources that will eventually be needed to support longer-term electrification. Specifically, resources like existing nuclear power plants may be at risk given their more limited operational flexibility, and with it, the inability to avoid low or negative-price market periods. Maintaining the portfolio of resources necessary to support decarbonization in this context will require additional products to be developed and monetized to compensate generators for when they are needed most.
From Storage to Balancing
In addition to the need to move more of the compensation of generators out of the traditional energy market and into capacity and flexibility markets, the nature of these will also have to be continuously evolving as the needs of the system evolve. As discussed in the previous section, the economic and reliability challenges of a highly renewable grid occur during net-load deficits and surpluses. Deficits occur when there are not enough renewables to meet load; surpluses occur when potential generation exceeds demand. These surpluses, with increasing penetrations of renewables, tend to persist over longer periods of time (e.g., solar overgeneration that might begin only at the peak of the day but start to extend over all daylight hours). Deficits, even with increasing penetrations of renewables, remain stubbornly long. Adding more and more renewables in fallow periods does little to address the problem. These persistent periods of renewable under-generation are often referred to with the German term Dunkelflaute. Meeting them requires generators with the ability to dispatch on a sustained basis, up to multiple days in a row.
​
The easiest technology to imagine performing this function is very long-duration energy storage, or multi-day energy storage (MDES) but this is not always the most cost-effective resource given its cost and projected inefficiencies. Backup thermal generators such as gas plants can also perform this function, limiting their generation to only the most critical periods. Resources like nuclear can be counted on to produce during these periods, but once constructed, they are economically operated at high-capacity factors. As a result, the issue of balancing between over-and under-generation of renewables is not ameliorated when they are operating as baseload resources.
​
Our model tends to solve these balancing challenges with a portfolio approach. We deploy electrolysis to operate during periods of plentiful renewables, reducing the overgeneration hours of renewables and allowing more economic deployment (because the load has shaped itself against a renewable profile). This hydrogen resulting from electrolysis can be stored in low-cost underground reservoirs in lieu of electricity storage. In addition, we deploy electrified steam technologies that operate when there are sufficient renewables (and otherwise use fuel boilers), reducing the demands for electricity and addressing seasonal imbalances of load and supply. These electricity loads that operate seasonally mean that during periods of low renewable generation, they can be turned off, reducing the overall scale of the under-generation period. The remaining Dunkelflaute is addressed with long-duration storage, hydro, thermal plants (burning zero-carbon fuels if necessary), and potentially the intelligent scheduling of nuclear maintenance so that fewer nuclear plants are offline during winter periods.​
The figure below shows both the overall increase in the need for balancing energy at different timescales as we move towards higher renewable systems as well as the contribution to balancing over- and under-generation at different timescales made by each resource type. Specific note should be paid to the increase in hourly balancing (principally driven by the solar overgeneration in the middle of the day) which is solved with electrolysis, short-duration battery storage, and flexible loads like industrial electrification and smart EV charging. Monthly balancing increases even more dramatically and this is where we see the impact of electrolysis (downward balancing during periods of over-generation) and the thermal fleet (upward balancing during periods of under-generation) in addressing the monthly imbalances in net load.
Report Materials
Interactive Results
